Sydney R. Stein, Sabrina C. Ramelli, Alison Grazioli, Joon-Yong Chung, Manmeet Singh, Claude Kwe Yinda, Clayton W. Winkler, Junfeng Sun, James M. Dickey, Kris Ylaya, Sung Hee Ko, et. al. Nature volume 612, pages758–763 (2022)Cite this article
Abstract
Coronavirus disease 2019 (COVID-19) is known to cause multi-organ dysfunction1,2,3 during acute infection with severe acute respiratory syndrome coronavirus 2 (SARS-CoV-2), with some patients experiencing prolonged symptoms, termed post-acute sequelae of SARS-CoV-2 (refs. 4,5). However, the burden of infection outside the respiratory tract and time to viral clearance are not well characterized, particularly in the brain3,6,7,8,9,10,11,12,13,14. Here we carried out complete autopsies on 44 patients who died with COVID-19, with extensive sampling of the central nervous system in 11 of these patients, to map and quantify the distribution, replication and cell-type specificity of SARS-CoV-2 across the human body, including the brain, from acute infection to more than seven months following symptom onset. We show that SARS-CoV-2 is widely distributed, predominantly among patients who died with severe COVID-19, and that virus replication is present in multiple respiratory and non-respiratory tissues, including the brain, early in infection. Further, we detected persistent SARS-CoV-2 RNA in multiple anatomic sites, including throughout the brain, as late as 230 days following symptom onset in one case. Despite extensive distribution of SARS-CoV-2 RNA throughout the body, we observed little evidence of inflammation or direct viral cytopathology outside the respiratory tract. Our data indicate that in some patients SARS-CoV-2 can cause systemic infection and persist in the body for months.
Main
COVID-19 has respiratory and non-respiratory manifestations1,2,3, including multi-organ failure and shock among patients with severe and fatal disease. Some individuals who survive experience post-acute sequelae of SARS-CoV-2, also known as long COVID4,5. Although autopsy studies of fatal COVID-19 cases support the ability of SARS-CoV-2 to infect multiple organs3,7,8,9,10,11,12, extrapulmonary organs often lack histopathological evidence of virally mediated injury or inflammation10,11,12,13,14. The paradox of infection outside the respiratory tract without injury or inflammation raises many pathogen- and host-related questions.
To investigate the cellular tropism, replication competence, persistence and evolution of SARS-CoV-2 in humans, and to look for associated histopathology in infected tissues, we carried out autopsies on 44 COVID-19 cases. Our approach focused on timely, systematic and comprehensive tissue sampling and preservation for complementary analyses. We carried out droplet digital polymerase chain reaction (ddPCR) for detection and quantification of SARS-CoV-2 nucleocapsid (N) gene targets and in situ hybridization (ISH) to validate the ddPCR findings and determine the cellular tropism of SARS-CoV-2. Immunofluorescence (IF) and chromogenic immunohistochemistry (IHC) were used to further validate the presence of SARS-CoV-2 in the brain. We carried out quantitative real-time PCR with reverse transcription (RT–qPCR) to detect subgenomic RNA, a marker suggestive of recent virus replication15, and demonstrated replication-competent SARS-CoV-2 in selected respiratory and non-respiratory tissues, including the brain, by virus isolation in traditional and modified Vero E6 cell culture. In six individuals, we measured the diversity and anatomic distribution of intra-individual SARS-CoV-2 spike gene variants using high-throughput, single-genome amplification and sequencing (HT-SGS).
We categorized autopsy cases as early (n = 17), mid (n = 13) or late (n = 14) by illness day (d) at the time of death, being ≤d14, d15–30 or ≥d31, respectively. We defined persistence as the presence of SARS-CoV-2 RNA among late cases. We analysed and described our results in terms of respiratory and non-respiratory tissues to quantify and statistically compare SARS-CoV-2 RNA levels across tissues and cases.
Autopsy cohort overview
Between 26 April 2020 and 2 March 2021, we carried out 44 autopsies, all among unvaccinated individuals who had died with COVID-19. SARS-CoV-2 PCR positivity was confirmed premortem in 42 cases and postmortem in 2 cases (P3 and P17; Extended Data Fig. 1). A total of 38 cases were SARS-CoV-2 seropositive (Supplementary Data 1a), 3 were seronegative (P27, P36 and P37), and plasma was unavailable for 3 cases (P3, P4 and P15). Brain sampling was accomplished in 11 cases (Fig. 1). The cohort was racially and ethnically diverse. Thirty per cent were female, and the median age was 62.5 years (interquartile range (IQR): 47.3–71.0; Extended Data Table 1a). A total of 61.4% had three or more comorbidities. The median interval from symptom onset to final hospitalization and subsequently death was 6 days (IQR: 3–10) and 18.5 days (IQR: 11.25–37.5), respectively (Extended Data Table 1b). The median postmortem interval was 22.2 h (IQR: 18.2–33.9). Individual-level case data can be found in Supplementary Data 2a.
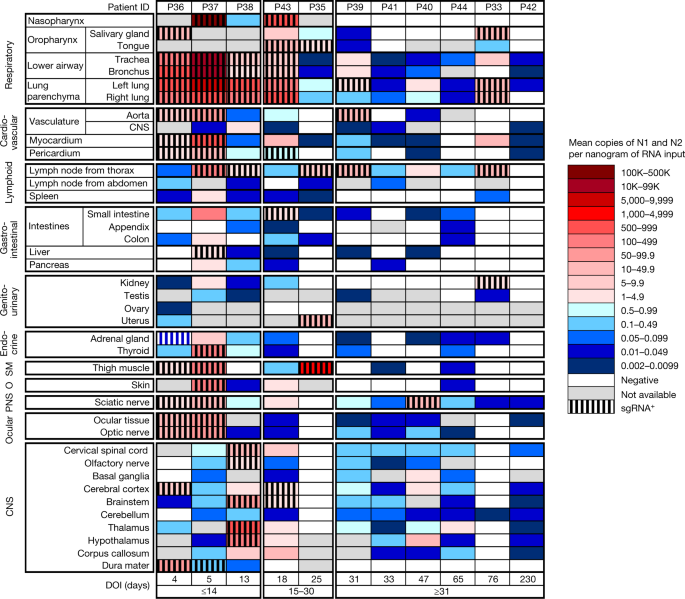
Widespread infection and persistence
SARS-CoV-2 RNA was detected in 84 distinct anatomical locations and body fluids (Supplementary Data 1b–d), with a significantly (P < 0.0001 for all) higher burden detected in respiratory compared with non-respiratory tissues among early (2.04 ± 0.10 log10[N gene copies] per nanogram of RNA), mid (1.36 ± 0.12 log10[N gene copies] per nanogram of RNA) and late (0.67 ± 0.11 log10[N gene copies] per nanogram of RNA; Extended Data Fig. 2a) cases. We compared linear trends in SARS-CoV-2 RNA levels by illness day, as a continuous variable, and observed a significantly steeper negative slope of SARS-CoV-2 RNA levels in respiratory (−3.14, s.e. 0.39) compared with non-respiratory (−1.62, s.e. 0.38; P < 0.0001) tissues (Extended Data Fig. 2b,c).
We detected SARS-CoV-2 RNA in perimortem plasma of 11 early and 1 mid case (Supplementary Data 1b,d). SARS-CoV-2 RNA was undetectable or just above the limit of detection in peripheral blood mononuclear cells from select early and mid cases (Supplementary Data 1a). The median and IQR of SARS-CoV-2 N gene copies per nanogram of RNA and proportion of cases with RNA detected in each tissue group and fluids are summarized in Extended Data Table 2. SARS-CoV-2 RNA persistence was detected across multiple tissue groups among all late cases despite being undetectable in plasma in any (Supplementary Data 1b–d). SARS-CoV-2 RNA was detected in central nervous system (CNS) tissue in 10/11 cases (90.9%), including across most brain regions evaluated in 5/6 late cases, including P42 who died at D230 (Fig. 1).
We detected SARS-CoV-2 subgenomic RNA across all tissue groups and multiple fluid types, including plasma, pleural fluid and vitreous humour (Supplementary Data 1a–c). ddPCR and subgenomic RNA RT-qPCR results closely correlated among 1,025 jointly tested samples (ρ = 0.76; 95% confidence interval (CI): 0.73–0.78), particularly among respiratory samples (n = 369, ρ = 0.86; 95% CI: 0.84–0.89), early cases (n = 496, ρ = 0.88; 95% CI: 0.85–0.89) and samples that tested positive by both assays (n = 302, ρ = 0.91; 95% CI: 0.88–0.93; Extended Data Fig. 2d,e). With sensitivity and specificity weighted equally, a ddPCR value of ≥1.47 N copies per nanogram of RNA predicted a positive subgenomic RNA result with 93.0% sensitivity and 91.6% specificity, with a receiver operating characteristic (ROC) area under the curve (AUC) of 0.965 (95% CI: 0.953–0.977; Extended Data Fig. 2f).
We isolated SARS-CoV-2 in Vero E6 cell culture from diverse tissues in and outside the respiratory tract including heart, lymph node, gastrointestinal tract, adrenal gland and eye from early cases (Extended Data Fig. 3a). In total, we isolated virus from 25/55 (45%) specimens tested across four SARS-CoV-2 subgenomic RNA quantification cycle (Cq) value intervals, with decreasing yield with rising Cq interval. Among the 55 samples tested for virus isolation, with sensitivity and specificity weighted equally, a ddPCR value of ≥758 N copies per nanogram of RNA predicted replication-competent virus with 76% sensitivity and 90% specificity (ROC AUC = 0.887; 95% CI: 0.795–0.978), and a subgenomic RNA value of ≥25,069 copies per microlitre of RNA (about Cq 22.40) predicted replication-competent virus with 72% sensitivity and 100% specificity (ROC AUC = 0.915; 95% CI: 0.843–0.987; Extended Data Fig. 3b,c).
We reattempted virus isolation from thalamus and hypothalamus of P38 on Vero E6-TMPRSS2-T2A-ACE2 cells16 and observed a cytopathic effect 48 h after inoculation with thalamus tissue homogenate from P38. RT–qPCR for SARS-CoV-2 envelope (E) genomic RNA was carried out on the tissue homogenate and the supernatant of the virus isolation process at the time of the cytopathic effect, and yielded Ct values of 27.33 and 13.24, respectively.
Viral genome sequencing
We used HT-SGS to analyse SARS-CoV-2 spike gene variant sequences from a total of 46 tissues in 6 individuals (Extended Data Fig. 4). In P27 (D1), P19 (D7) and P18 (D9), no nonsynonymous virus genetic diversity was detected in respiratory and non-respiratory sites despite a high depth of single-molecule sampling. In P27, two virus haplotypes, each with a single synonymous substitution, were preferentially detected in non-respiratory sites including the right and left ventricles and mediastinal lymph node. In P38 (D13), a D80F residue was identified in 31/31 pulmonary but 0/490 brain sequences, and a G1219V residue was restricted to brain variants. SARS-CoV-2 virus isolated from thalamus of P38 through Vero E6-TEMPRSS2-TA2-ACE2 cell culture and subjected to short-read, whole-genome sequencing matched the minor haplotype detected from P38 RNAlater-preserved thalamus and the major haplotype of P38 RNAlater-preserved hypothalamus. A nonsynonymous substitution was also detected in P36 (D4) dura mater, albeit at very low sampling depth (n = 2 sequences), compared with non-CNS tissue.
ISH reveals the cellular tropism of SARS-CoV-2
We validated our ddPCR results by ISH for SARS-CoV-2 spike RNA in respiratory and non-respiratory tissues in selected early, mid and late cases across >35 cell types and hyaline membranes (Figs. 2 and 3, Extended Data Table 3 and Supplementary Data 3). Detailed annotation of SARS-CoV-2 spike RNA ISH-positive cells by tissue, including across multiple brain regions, is provided in Figs. 2 and 3 and Supplementary Data 3.
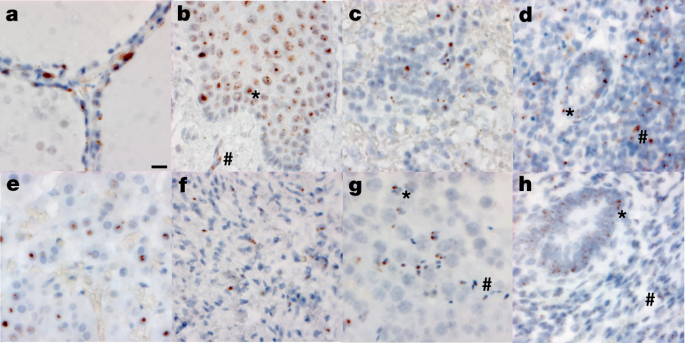
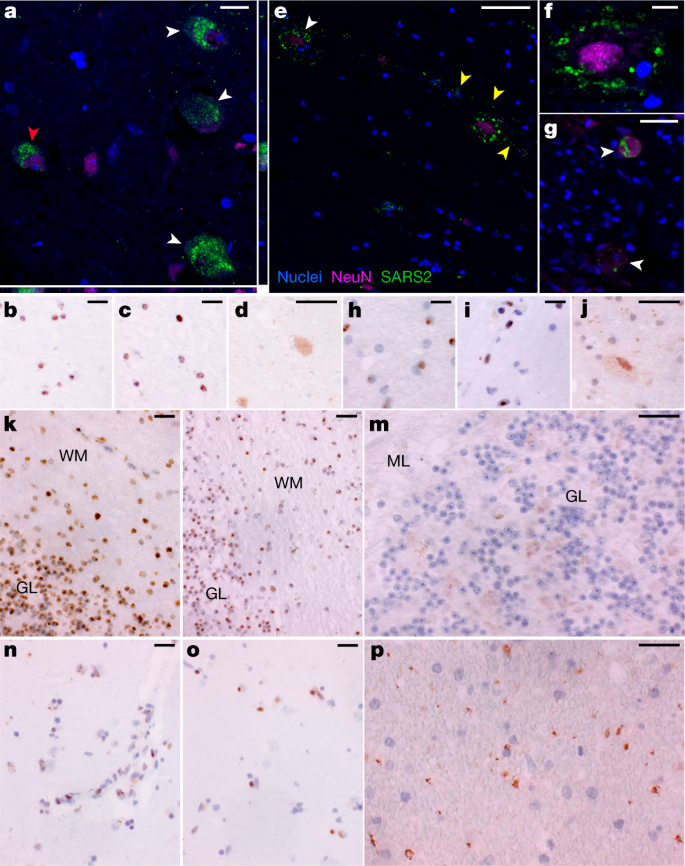
To determine the relationship between SARS-CoV-2 N RNA detected by ddPCR and SARS-CoV-2 spike RNA detected by ISH, we carried out image analysis on interventricular septal tissue from 16 cases covering a four-log range of SARS-CoV-2 N gene copies per nanogram of RNA ddPCR values. Interventricular septum was selected for this analysis owing to consistent histomorphology. Mean SARS-CoV-2 N gene copies per nanogram of RNA significantly correlated with the median SARS-CoV-2 spike RNA-positive cells over thirty ×40 fields (ρ = 0.704, 95% CI: 0.320–0.889, P = 0.002; Supplementary Data 3).
SARS-CoV-2 N RNA and protein in CNS
To further validate detection and distribution of SARS-CoV-2 in CNS tissue, we used a second ISH assay targeting N RNA, and IF and chromogenic IHC-based assays targeting N protein. We confirmed the specificity of these assays with appropriate positive and negative controls (Supplementary Data 3, panels yy–bbb) and applied them to selected CNS tissues that were SARS-CoV-2 positive by ddPCR. We observed SARS-CoV-2 RNA and protein in hypothalamus and cerebellum of an early case (P38) and cervical spinal cord and basal ganglia of late cases (P42 and P40, respectively), with a pattern consistent with neuronal staining (Fig. 3).
COVID-19 histological findings
The histopathology findings from our cohort were similar to those reported in other case series (Extended Data Fig. 5). Of 44 cases, 38 were determined to have died from COVID-19, and of these, 35 (92.1%) had either acute pneumonia or diffuse alveolar damage at the time of death (Supplementary Data 2). Phases of diffuse alveolar damage showed a clear temporal progression (Extended Data Fig. 6). Pulmonary thromboembolic complications were noted in 10 (23%) cases and myocardial infiltrates were observed in four cases, including one case of substantial myocarditis17 (P3). In the lymph nodes and spleen, we observed both lymphodepletion and follicular and paracortical hyperplasia.
Other non-respiratory histological changes were mainly related to complications of therapy or pre-existing comorbidities. Five cases had old ischaemic myocardial scars and three had coronary artery bypass grafts in place. Diabetic nephropathy and steatohepatitis were observed in ten cases (23%) and five cases (12%), respectively. One case had known hepatitis C with cirrhosis, but the other cases of advanced hepatic fibrosis were probably related to fatty liver disease. Hepatic necrosis (13 cases, 30%) and changes consistent with acute kidney injury (17 cases, 39%) were probably related to hypoxic–ischaemic injury in these very ill patients.
In the examination of 11 brains, we found few histopathologic changes, despite substantial viral burden. Vascular congestion was an unusual finding that had an unclear aetiology and could be related to the haemodynamic changes incurred with infection. Global hypoxic–ischaemic change was seen in two cases, one of which was a juvenile (P36) with a seizure disorder who was found to be SARS-CoV-2 positive on hospital admission, but who probably died of seizure complications unrelated to viral infection.
Discussion
Here we provide, to our knowledge, the most comprehensive analysis to date of the cellular tropism, quantification and persistence of SARS-CoV-2 across the human body including the brain. Our focus on short postmortem intervals, a comprehensive standardized approach to tissue collection, dissecting the brain before fixation, preserving tissue in RNAlater and flash freezing of fresh tissue allowed us to detect and quantify SARS-CoV-2 RNA levels with high sensitivity by ddPCR and ISH, as well as isolate virus in cell culture from multiple non-respiratory tissues including the brain, which are notable differences compared to other studies.
We show that SARS-CoV-2 disseminates early in infection in some patients, with a significantly higher viral burden in respiratory than non-respiratory tissues. We demonstrated virus replication in multiple non-respiratory sites during the first two weeks following symptom onset and detected subgenomic RNA in at least one tissue in 14 of 27 cases beyond D14, indicating that viral replication may occur in non-respiratory tissues for several months. Whereas others have proposed that the detection of SARS-CoV-2 in non-respiratory tissues might be due to residual blood within tissues8,18 or cross-contamination from the lungs during tissue procurement8, our data indicate otherwise. Specifically, only 12 of our cases had detectable SARS-CoV-2 RNA in a perimortem plasma sample, only 2 cases had SARS-CoV-2 subgenomic RNA detected in plasma, and negligible, if any, RNA was detected in banked peripheral blood mononuclear cells from representative cases. Further, we validated detection of SARS-CoV-2 outside the respiratory tract by direct cellular identification of virus in cells through ISH, IHC and IF, isolation of SARS-CoV-2 by cell culture, and detection of distinct SARS-CoV-2 spike sequence variants in non-respiratory sites.
Others have previously reported SARS-CoV-2 RNA within the heart, lymph node, small intestine and adrenal gland6,8,9,10,11,12,18. We replicate these findings and conclusively demonstrate that SARS-CoV-2 is capable of infecting and replicating within these and many other tissues, including brain. Specifically, we report the recovery of replication-competent SARS-CoV-2 from thalamus of P38 at D13 using a modified Vero E6 cell line that stably expresses ACE2 and TMPRSS2. This along with detection of genomic RNA and subgenomic RNA through PCR, multiple imaging modalities showing SARS-CoV-2 RNA and protein within cells of the CNS, and distinct minor variants detected through sequencing in the CNS prove definitively that SARS-CoV-2 is capable of infecting and replicating within the human brain.
HT-SGS of SARS-CoV-2 spike demonstrates homogeneous virus populations in many tissues, while also revealing informative virus variants in others. Low intra-individual diversity of SARS-CoV-2 sequences has been observed frequently in previous studies19,20,21, and probably relates to the intrinsic mutation rate of the virus as well as lack of early immune pressure to drive virus evolution. It is important to note that our HT-SGS approach has both a high accuracy and a high sensitivity for minor variants within each sample, making findings of low virus diversity highly reliable22. Genetic compartmentalization of SARS-CoV-2 between respiratory and non-respiratory tissues in several individuals supports independent replication of the virus at these sites, although lack of compartmentalization between sites does not rule out independent virus replication. We note several cases in which brain-derived virus spike sequences showed nonsynonymous changes relative to sequences from other non-CNS tissues. Further studies will be needed to understand whether these cases might represent stochastic seeding of the CNS or differential selective pressure on spike by antiviral antibodies in the CNS, as others have suggested23,24,25.
Our results show that although the highest burden of SARS-CoV-2 is in respiratory tissues, the virus can disseminate throughout the entire body. Whereas others have posited that this viral dissemination occurs through cell trafficking11 due to a reported failure to culture SARS-CoV-2 from blood3,26, our data support an early viraemic phase, which seeds the virus throughout the body following infection of the respiratory tract. Recent work26 in which SARS-CoV-2 virions were pelleted and imaged from plasma of patients with acute COVID-19 supports this mechanism of viral dissemination. Our cohort is predominantly composed of severe and ultimately fatal COVID-19 cases. However, two cases (P36 and P42) reported only mild or no respiratory symptoms and died with, not from, COVID-19, yet had SARS-CoV-2 RNA widely detected across the body and brain. Additionally, P36 was a juvenile with an underlying neurological condition, but without evidence of multisystem inflammatory syndrome in children, suggesting that children may develop systemic infection with SARS-CoV-2 without developing a generalized inflammatory response.
Finally, our work begins to elucidate the duration and locations at which SARS-CoV-2 RNA can persist. Although the respiratory tract was the most common location in which SARS-CoV-2 RNA persisted, ≥50% of late cases also had persistent RNA in the myocardium, lymph nodes from the head and neck and from the thorax, sciatic nerve, ocular tissue, and in all sampled regions of the CNS, except the dura mater. Notably, despite having more than 100 times higher SARS-CoV-2 RNA in respiratory compared to non-respiratory tissues in early cases, this difference greatly diminished in late cases. Less efficient viral clearance in non-respiratory tissues may be related to tissue-specific differences in the ability of SARS-CoV-2 to alter cellular detection of viral mRNA, interfere with interferon signalling, or disrupt viral antigen processing and presentation27,28,29. Understanding mechanisms by which SARS-CoV-2 evades immune detection is essential to guide future therapeutic approaches to facilitate viral clearance.
We detected subgenomic RNA in tissue from more than 60% of the cohort, including in multiple tissues of a case at D99. Although subgenomic RNA is generated during active viral replication, it is less definitive than cell culture at demonstrating replication-competent virus because subgenomic RNA is protected by double-membrane vesicles that contribute to nuclease resistance and longevity beyond immediate viral replication30,31,32,33. However, nonhuman primates exposed to γ-irradiated SARS-CoV-2 inoculum with high subgenomic RNA copy numbers through multiple mucosal routes had detectable SARS-CoV-2 genomic RNA but undetectable subgenomic RNA levels in respiratory samples by day 1 post-inoculation15. These data suggest that detection of SARS-CoV-2 subgenomic RNA probably reflects recent viral replication. Prolonged detection of subgenomic RNA in a subset of our cases may, however, represent defective rather than productive viral replication, which has been described in persistent infection with measles virus—another single-strand enveloped RNA virus—in cases of subacute sclerosing panencephalitis34.
Our study has several important limitations. First, our cohort largely represents older unvaccinated individuals with pre-existing medical conditions who died from severe COVID-19, limiting our ability to extrapolate findings to younger, healthier or vaccinated individuals. Second, our cases occurred during the first year of the pandemic, before widespread circulation of variants of concern, and thus findings might not be generalizable to current and future SARS-CoV-2 variants. Finally, although it is tempting to attribute clinical findings observed in post-acute sequelae of SARS-CoV-2 to viral persistence, our study was not designed to address this question. Despite these limitations, our findings fundamentally improve the understanding of SARS-CoV-2 cellular distribution and persistence in the human body and brain and provide a strong rationale for pursuing future similar studies to define mechanisms of SARS-CoV-2 persistence and contribution to post-acute sequelae of SARS-CoV-2.