David Sulzer, Angelo Antonini, Valentina Leta, Anna Nordvig, Richard J. Smeyne, James E. Goldman, Osama Al-Dalahmah, Luigi Zecca, Alessandro Sette, Luigi Bubacco, Olimpia Meucci, Elena Moro, Ashley S. Harms, Yaqian Xu, Stanley Fahn & K. Ray Chaudhuri
npj Parkinson’s Disease volume 6, Article number: 18 (2020) Cite this article
Abstract
This Viewpoint discusses insights from basic science and clinical perspectives on coronavirus disease 2019 (COVID-19)/severe acute respiratory syndrome-coronavirus-2 (SARS-CoV-2) infection in the brain, with a particular focus on Parkinson’s disease. Major points include that neuropathology studies have not answered the central issue of whether the virus enters central nervous system neurons, astrocytes or microglia, and the brain vascular cell types that express virus have not yet been identified. Currently, there is no clear evidence for human neuronal or astrocyte expression of angiotensin-converting enzyme 2 (ACE2), the major receptor for viral entry, but ACE2 expression may be activated by inflammation, and a comparison of healthy and infected brains is important. In contrast to the 1918 influenza pandemic and avian flu, reports of encephalopathy in COVID-19 have been slow to emerge, and there are so far no documented reports of parkinsonism apart from a single case report. We recommend consensus guidelines for the clinical treatment of Parkinson’s patients with COVID-19. While a role for the virus in causing or exacerbating Parkinson’s disease appears unlikely at this time, aggravation of specific motor and non-motor symptoms has been reported, and it will be important to monitor subjects after recovery, particularly for those with persisting hyposmia.
Introduction
Over the past twenty years, novel viral epidemics, including influenza, severe acute respiratory syndrome (SARS) and Middle Eastern respiratory syndrome (MERS), have appeared, likely through zoonosis1,2,3,4,5,6. There are few, if any, therapeutic options for treating these disorders and they can induce significant mortality7,8. In 2019, a novel coronavirus outbreak, known as COVID-19, was reported in China, and as of May 2020, it had spread to 229 countries9.
This coronavirus, known as SARS-CoV-2, is a large enveloped non‐segmented positive‐sense RNA virus10. When the SARS-CoV-2 virus, and in particular its Spike (S) protein, makes contact with cells, it binds to a number of host proteins (known as virus receptors) that assist in its entry10.
Symptoms
Like its related family members SARS-CoV and MERS-CoV, SARS-CoV-2 initially presents as a respiratory illness, characterized by cough, dyspnea, fever, and other upper and lower respiratory systems manifestations11. However, COVID-19 is associated with a variety of other symptoms and clinical manifestations due to its spread to many other organs and systems11.
At this time, it appears that all subjects who have recovered from COVID-19 have developed T cells that recognize specific viral epitopes, including the S protein12. The extraordinarily wide range of symptoms and severity, including many infected subjects showing mild or no effects, may be due to cross-reactivity of T cells previously developed in response to prior coronavirus infections that cross-react with SARS-CoV-2, and it is remarkable that nearly half of individuals tested from blood samples prior to 2019 have such cells12. It is also possible that different routes of infection, including via the gastrointestinal tract, may result in different symptoms13.
Epidemiological and public health studies indicate that infection with the SARS-CoV-2 affects all demographics, but has grave implications for older frail subjects14, particularly those with comorbidities as well as Black, Asian, and minority ethnic (BAME) subjects in a disproportionate manner (https://www.england.nhs.uk/coronavirus/workforce/addressing-impact-of-covid-19-on-bame-staff-in-the-nhs/). This is not always the case for viral disorders, as some, like polio, are typically more dangerous for the young15. The impression that SARS-CoV-2 infection was particularly pathogenic in older frail subjects has been confirmed by high mortality rates, particularly in residential home patients across the United Kingdom, Italy, the United States, and many other countries16,17. Moreover, other comorbidities and factors have been associated with more severe infection, such as diabetes, obesity, pre-existing end organ disease, hypertension, and male sex18,19. It has been suggested that the cytokine storm is more easily triggered in patients with chronic inflammation, such as those with diabetes, obesity, and cardiac disease20. The cause of high mortality in older BAME subjects reported in the UK and USA remains unclear, although role of comorbidities such as diabetes, hypertension, and obesity as well as social deprivation are implicated (https://www.england.nhs.uk/coronavirus/workforce/addressing-impact-of-covid-19-on-bame-staff-in-the-nhs/).
While the majority of infected people exhibit mild or moderate symptoms and do not require hospitalization, more severe patients need to be hospitalized and sometimes intubated due to severe respiratory distress21. Other serious consequences of COVID-19 include acute kidney injury, a coagulopathy similar to disseminated intravascular coagulation22, thrombosis23, and a newly recognized post-infection syndrome in children, known as multi-system inflammatory syndrome in children potentially associated with COVID-1924. The sequelae of each of these syndromes can result in multi-organ failure11,25.
A significant number of those diagnosed with COVID-19 have reported a broad spectrum of neurological consequences26,27,28,29,30,31,32. Neurological symptoms include those associated with dysfunction of the central (fatigue, headache, confusion, stroke33, dizziness, syncope34, seizure, anorexia, and insomnia)35,36,37,38, peripheral (anosmia, ageusia, myoclonus39, neuropathic pain, and myalgias)26,35,40, combined central-peripheral (Guillain Barre syndrome41) and enteric nervous systems (diarrhea13). Some gastrointestinal manifestations, including diarrhea, may be related to the expression of the viral receptor ACE2 and a serine protease, transmembrane serine protease 2 (TMPRSS2), involved in S protein priming, in the small intestinal epithelia and colon42.
As many as 65% of COVID-19 affected individuals reported hyposmia and ageusia43, features that suggest the possibility of trans-synaptic spread via the olfactory, lingual, and glossopharyngeal nerves (Fig. 1), secondary to a respiratory route of infection. Hyposmia is now officially recognized as a symptom of COVID-19 by the UK government and may be a sign in “asymptomatic” carriers who may not have upper respiratory tract symptoms.
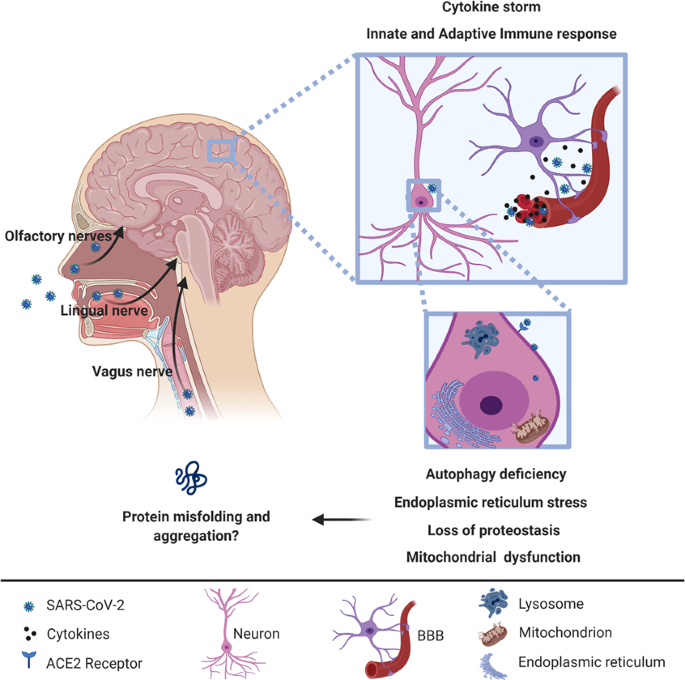
A recent review of 43 confirmed COVID-19 cases in a London, UK hospital suggested emergence of specific neurological presentations, including encephalopathies, inflammatory central nervous system syndromes, ischemic strokes, and peripheral neurological disorders, although parkinsonism and rates of hyposmia or ageusia were not reported44.
We and others have previously flagged concerns regarding COVID-19 in people with Parkinson’s disease (PD), especially for older and frail subjects with advanced PD who may be particularly vulnerable45,46.
Historical aspects of viruses and parkinsonism
It is remarkable that a relationship between the presence of antibodies to coronaviruses that cause the common cold, coronavirus OC43 and 229E, in the cerebrospinal fluid (CSF) and Parkinson’s disease was reported nearly twenty years prior to the current pandemic by Stanley Fahn and colleagues47. Prior coronaviruses have been occasionally reported to exhibit neurological manifestations and CSF invasion48, including in children49,50.
Medical history provides observations supporting links between viral infections and parkinsonism51. The best known example is the post-encephalitic parkinsonism observed during the encephalitic lethargica outbreak that overlapped with the Spanish Flu (influenza A virus H1N1) pandemic in 191852. However, after 100 years, the cause of encephalitis lethargica still remains a mystery53. While a causal role of influenza A H1N1 virus in the development of post-encephalitic parkinsonism is not confirmed52, an association between influenza A virus infection and development of transient parkinsonism is reported54. Notably, the avian flu resulted in parkinsonism in many survivors55. Other viral infections have been associated with the development of transient or, more rarely, permanent parkinsonism, including Epstein-Barr, Japanese encephalitis, Coxsackie, West Nile, Western equine encephalomyelitis, and human immunodeficiency virus, mostly due to induction of neuroinflammation and/or hypoxic brain injury with structural/functional damage within the basal ganglia51,54 (Table 1). In addition, debated evidence suggests that previous infection with herpes simplex 1, Epstein-Barr, varicella zoster, hepatitis C, and influenza A virus can increase the risk of developing PD in the long-term54. Although the “viral hypothesis“ was generally ignored after the discovery of genetic mutations involved in PD pathogenesis, the role of “environmental” factors acting as peripheral triggers of the neurodegenerative process in susceptible individuals has been increasingly acknowledged56.Table 1 Mechanisms involved in the pathogenesis of viral-induced parkinsonism.
SARS-CoV-2 receptors and cellular uptake
There is a wide diversity of proteins, particularly glycoproteins, that act as cellular receptors for coronavirus spike proteins57.
SARS-CoV-2 shares 70–80% of its genome with SARS-CoV and a smaller but significant homology with MERS-CoV58. This homology extends to the S protein58 that is the point of attachment to plasma membrane proteins which act as viral receptors for cellular infection. The S protein is thought to require a priming step in which it is cleaved by a cellular protease, which for SARS-CoV and SARS-CoV-2 is reported to be the cellular serine protease, TMPRSS259.
The extensive research devoted to determining how the binding of the virus leads to cellular endocytosis of the virus, leading ultimately to RNA translation, transcription, and viral replication, will not be reviewed here.
At this time it appears that the main protein responsible for cellular accumulation of SARS-CoV-2 is angiotensin-converting enzyme 2 (ACE2)27,60,61, an enzyme that converts angiotensin II to angiotensin. ACE2 also acts as a receptor for several other coronavirus, including SARS-CoV62,63. The distribution of ACE2 throughout the body and brain is discussed below.
Recent in-silico studies64,65 propose that, in addition to ACE2, a second mechanism may enable cellular endocytosis of SARS-CoV-2. Similar to MERS-CoV, and in contrast to SARS-CoV, SARS-CoV-2 appears to display high binding affinity to sialic acid residues, providing an additional candidate for binding. Sialic acid residues are found on plasma membrane proteins of many cell types, including neurons, and are very highly expressed in the upper respiratory tract.
Additional observations that may test the predictions from in-silico reports implicating a role for sialic acid residues as SARS-CoV-2 receptors include (1) efficacy of the therapeutic use of lactoferrin66, an antiviral agent that interacts with sialic acid residues; (2) an ongoing clinical trial of DAS181 (https://clinicaltrials.gov/ct2/show/NCT04324489), a drug designed to block viral access by cleaving sialic acid; (3) that the shedding pattern of SARS-CoV-2 infection is different from that of SARS-CoV and more similar to that of “standard” influenza66, where sialic acid receptors play a major role; (4) a bioinformatic study reports binding of S-protein to sialic acid glycans in a region close to that identified by the in silico studies65,67. To our knowledge, while links between sialic acid and neurotropism for mouse hepatitis virus68 and adenoviruses69 have been suggested, there are no published investigations on this alternative pathway for SARS-CoV-2 interaction in the nervous system.
There are additional strong candidates for receptors for the virus, including the lectin CD209L (also known as L-SIGN), which acts as a receptor for the SARS virus62,70. This should be analyzed both in the nervous system and additional tissues, as well as other suggested candidate coronavirus receptors, most of which are highly charged and glycosylated57.
Potential neurotropism of COVID-19 virus
At this time, we know very little about SARS-CoV-2 in the brain. Post-mortem studies on patients with SARS, however to have suggested the presence of viral particles in central nervous system (CNS) tissue71,72.
A recent publication examining the localization of SARS-CoV-2 in 27 people who died from COVID-19 demonstrated that 36% had apparently low levels of SARS-CoV-2 RNA and proteins in the brain, although they did not report the cellular localization or regions examined, and the signals may not have been present within the brain parenchyma73. A second study similarly reports detectable SARS-CoV-2 RNA in four of 12 brain samples, although again the signal may not have been from brain parenchymal cells74.
While there is, at this time, little evidence that SARS-CoV-2 enters the brain parenchyma, there are multiple means by which the virus might be able to do so75. Preclinical animal studies (reviewed by Natoli et al.76) report that after intranasal inoculation of SARS‐CoV in transgenic mice that overexpress human ACE277, or MERS-CoV in mice overexpressing human dipeptidyl peptidase 478, SARS‐CoV and MERS-CoV can invade the brain, possibly via transit through the olfactory nerves, to reach CNS nuclei, including thalamus and brainstem; we note, however, that these mice over-express the viral receptors, and these reports do not model normal infection routes.
Trans-synaptic transfer has been documented in rat and pig for other types of coronavirus, including hemagglutinating encephalomyelitis virus (HEV)79,80,81 and avian infectious bronchitis virus (IBV, also known as avian coronavirus)82, in both in vitro and in vivo studies.
Coronavirus might also reach the CNS via the hematogenous or lymphatic route, although this seems unlikely in early phases of the disease, as particles of SARS-CoV were not detected in non-neuronal cells in human post-mortem brain tissue71,72.
One potential mechanism for SARS-CoV-2 RNA presence within the CNS is blood-brain barrier (BBB) breakdown due to the cytokine storm associated with peripheral viral infection. It is well established that pro-inflammatory cytokines associated with inflammation and/or SARS-CoV-2 viral infection, such as tumor necrosis factor (TNF) and interleukin 1 beta (IL-1beta), mediate BBB breakdown83. This breakdown could either be long-term, similar to the one observed in neurodegenerative diseases allowing for infiltration of immune cells and viral particles, or transient, resulting in encephalitis84,85.
We note that while there are at this time several millions of SARS-CoV-2 infected individuals, there are only a few reports suggesting possible encephalitis, and only two that show evidence of COVID-19 virus in the CSF as assessed by reverse transcription polymerase chain reaction (RT-PCR). This suggests that even with the presence of high viral load in the blood stream and severe inflammation, COVID-19 virus is unlikely to exhibit direct neurotropism, but rather appears to cause inflammatory-mediated brain responses86.
Presence of SARS-CoV-2 receptors in the brain
ACE2 was identified in 2000 as a novel carboxypeptidase that cleaves the vasoconstrictor angiotensin II to the vasodilator angiotensin (1–7), in addition to cleaving several other peptides87. ACE2 is a transmembrane protein, and can itself be cleaved near the transmembrane region and thereby be “shed” into a soluble form with anti-viral activity88,89, in part as the soluble form likely binds virus. Plasma membrane ACE2 is, confusingly, often referred to as the “ACE2 receptor”, but this is intended to convey that the protein, in addition to its normal function, can act as a receptor for virus—and not that it is a receptor for ACE2.
ACE2 is widely expressed in human tissue90 and appears to be increased by inflammatory signals including in macrophages91. Evidence supporting ACE2 expression in human brain parenchyma, however, remains poor, in contrast to clear expression in the brain vessels92. There is an extensive literature indicating that ACE2 may serve as a protective stress-induced response pathway93,94,95 and that its expression might be harnessed clinically for cardiac and neurological disorders, which will not be reviewed here.
In particular, while ACE2 expression has been demonstrated in CNS neurons in some animal models96,97, the presence of ACE2 in human CNS neurons is not well established, nor are specific brain regions or neuronal, astrocyte, microglial, immune or vascular cell types well characterized.
The ACE2 promoter harbors five hypoxia-responsive elements, and hypoxia may upregulate ACE2 via HIF1A-independent mechanisms98, but it has not yet been determined if hypoxia upregulates ACE2 in brain cells.
It is very important to compare the presence of brain ACE2, and perhaps of CD209L and molecules with sialic acid residues, in both control individuals and those with high inflammation. The expression of some of these “receptors”, including ACE2, can be enhanced by cytokines, such as interferon99, or other inflammatory responses90, and may be regulated by excitotoxicity100.
The Human Protein Atlas reports that ACE2 is not detected in normal human brain, but indicates low amounts in mouse brain (https://www.proteinatlas.org/ENSG00000130234-ACE2/tissue). As mentioned, an immunocytochemistry study of human brain tissue indicated that ACE2 is present in non-neuronal cells of vasculature in human brain tissue92, although that study did not define the precise cell types that express the receptor. A preprint of a single cell transcriptomic analysis suggests differential levels of ACE2 mRNA in different mouse brain regions101. Another preprint features a meta-analysis of single-cell and single-nucleus RNA sequencing datasets indicating co-expression of ACE2 and TMPRSS2 in oligodendrocytes102. However, additional studies are required to validate and localize protein co-expression in the CNS.
Because SARS-CoV-2 proteins can interact with host proteins involved in pathways that are altered during aging, including potential mitochondrial dysfunction, loss of proteostasis, autophagy dysfunction, inflammation, and endoplasmic reticulum stress, it is possible that SARS-CoV-2 infection may prompt protein misfolding and aggregation (Fig. 1)103,104,105. Of particular relevance for PD, recent studies have suggested that the aggregation-prone protein, alpha-synuclein, plays a role in the innate immune response to viral infections106,107.
It will be important to follow up and clinically monitor patients infected by COVID-19 virus, particularly those who developed specific neurological disturbances, such as sustained hyposmia108, syncope, and persistent confusion, given the relevance of these conditions to PD and PD dementia. Hyposmia is a well-recognized prodromal feature of PD109 as well as Alzheimer’s disease110 and may be due in part to dysfunction of inhibitory dopaminergic neurons in the olfactory bulb111. Although we do not yet know the precise mechanisms underlying hyposmia in COVID-19, it may be that patients who develop hyposmia become more susceptible to a neurodegenerative process or, alternatively, hyposmia may be a sign of peripheral inflammatory involvement of the olfactory mucosa. It is, therefore, reasonable to suggest specifically following up those COVID-19-linked cases where recovery is associated with sustained hyposmia after the acute illness of COVID-19 has subsided.
COVID-19 and the possibility of a post-viral parkinsonism: clinical and molecular rationales
Some literature has already highlighted potential links between COVID-19 virus and neurodegenerative conditions, including suggestions regarding PD104,112. These are based on multiple observations:
- 1.The ability of coronaviruses to enter the CNS through the nasal cavity with subsequent neuronal death77,78, as shown in animal studies.
- 2.Hyposmia is well documented in COVID-19 patients without nasal obstruction and rhinorrhea108,113,114 and is also a common prodromal feature of PD115.
- 3.Basal ganglia lesions may occur in the context of a thromboembolic encephalopathy in COVID-19116.
- 4.The presence of higher levels of antibodies against other coronaviruses that cause the common cold in the CSF of PD patients compared to healthy controls suggests a possible involvement of viral infection in the pathogenesis of PD47.
- 5.There are reports that ACE2 may be expressed in various regions of the nervous system93,117, although as detailed above, further neuropathological investigation is required. Given the interferon activation of this protein, it will be important to examine subjects with CNS inflammation or encephalitis.
- 6.The recent reports of syncope with no abnormal rhythms on cardiac device interrogation hint at a potential role for neurally-mediated syncope34 vs. orthostasis, suggesting the importance of these investigations for PD patients who often suffer from dysautonomia118.
- 7.A single case report of a patient who developed myoclonus and an acute but spontaneously reversible hypokinetic rigid syndrome, with DaTscan showing reduction of dopamine transporter uptake in the putamen as well as hyposmia119.
- 8.The angiotensin system, which is implicated in COVID-19 pathogenesis, may be important in neuroinflammatory and neurodegenerative mechanisms observed in PD120,121.
- 9.SARS-CoV-2 proteins can interact with human proteins involved in biological mechanisms that drive dysfunction of protein homeostasis that may lead to protein misfolding and aggregation (Fig. 1)103,104.
- 10.The release of cytokines may activate resident immune cells in the CNS and/or lead to their infiltration from the periphery that result in brain cell damage. Such cells may include activated T cells and microglia that may kill neurons122,123,124, astrocytes, and vascular cell types. This may occur through the selection of cells that specifically recognize presented antigens from the infection or previous infections, or via a general activation of cytotoxic cells that recognize other antigens, including autoantigens, such as those derived from alpha-synuclein which are implicated in PD, Lewy Body dementias, multiple system atrophy, and multiple sclerosis125,126. High levels of pro-inflammatory cytokines, such as TNF and IL-1beta, are associated with increased risk of PD, while use of non-steroidal anti-inflammatory drugs (NSAIDs) and anti-TNF biologics reduce the risk127. Anti-TNF biologics are currently under investigation for COVID-19.
Beyond the significant observational literature discussed above that suggests a relationship between viral infection and PD51, a number of preclinical studies have directly addressed this issue. Jang et al. examined the potential for a neurotropic Type A influenza virus (A/Vietnam, 1203/04, H5N1, a.k.a. bird flu) to induce parkinsonian pathology in mice. They found that this strain of influenza virus directly infected neurons, with particular affinity for circuits involved in PD. Subsequent to recovery from this infection, the mice exhibited ataxia, tremor, and bradykinesia128 as well as a transient but significant loss of dopaminergic neuron phenotype, an early neuroinflammatory program, long-lasting microgliosis and an increase in alpha-synuclein expression129.
Another neurotropic virus, the mosquito-borne alphavirus, Western equine encephalitic virus (WEEV) also induces post-encephalitic parkinsonism. Like the influenza virus, WEEV induced activation of microglia and astrocytes, selective loss of dopaminergic neurons in the substantia nigra pars compacta (SNpc) and behavioral abnormalities consistent with PD in mouse models130. Importantly, the common denominator of these viruses is that they enter the CNS and directly infect cells.
As we do not yet know if the SARS-CoV-2 virus directly infects CNS neurons, it is important to determine if non-neurotropic viruses also have the potential to contribute to development of PD. The idea that a peripheral cytokine storm from non-neurotropic viruses can induce encephalitis has been suggested for many other viral infections, including the 1918 Spanish influenza (Type A H1N1)131,132 as well as respiratory syncytial virus133.
Notably, the pandemic 2009 H1N1 (CA/09) influenza virus does not infect neurons in the central, peripheral or enteric nervous systems, but can nevertheless induce a significant inflammatory response in the CNS, including within the SNpc. Evidence that an indirect neuroinflammatory mechanism of this sort might increase the risk of parkinsonism is that mice infected with the 2009 H1N1 virus, after complete resolution of peripheral infection, displayed a higher level of SNpc DA neuron death after injection with the parkinsonian neurotoxin, 1-methyl-4-phenyl-1,2,3,6-tetrahydropyridine (MPTP). Administration of an influenza vaccine or the neuraminidase inhibitor oseltamivir (Tamiflu) protected against the synergistic response to the neurotoxin134. In these preclinical studies, microgliosis and increase in inflammatory cytokines and chemokines in the brain were not due to invasion of CD4+/CD8+ T-cells from the periphery, suggesting that inflammatory cytokines released during peripheral infection passed through the blood-brain barrier135 and indirectly activated microglia, leading to a parkinsonian cascade.
Interestingly, influenza vaccination in humans enhanced levels of the anti-inflammatory cytokine interleukin 10 (IL-10)136, while prophylactic treatment with oseltamivir (Tamiflu) decreased disease severity of influenza in both human and mouse models, and did not appear to interfere with appropriate T cell responses to new influenza infection137. It may be that vaccination is protective for nervous system inflammatory responses even from viruses that do not infect neurons and astrocytes.
The need for detailed autopsy studies
As deaths from SARS-CoV-2 infection continue, autopsy studies will play a key role in defining CNS pathology, including in patients with PD. However, due to increased precautions taken at the time of autopsy, relatively few brain autopsies are being performed. The U.S. Centers for Disease Control has issued guidance on autopsies for confirmed SARS-CoV-2 decedents and advises against performing procedures that generate aerosols, such as those used to remove the brain (https://www.cdc.gov/coronavirus/2019-ncov/hcp/guidance-postmortem-specimens.html#biosafety). Most studies thus far lack neuropathologic characterization altogether138,139,140,141,142, and an autopsy case series did not provide detailed neuropathologic descriptions74. Moreover, deaths occurring in nursing home and long-term care facilities, where a large subset of patients suffering from dementia reside, are less likely to result in autopsies. We thus expect a delay in understanding whether and how SARS-CoV-2 infection specifically alters neuropathology, including in PD.
Of the available studies with some neuropathologic data, one case series of ten autopsies documented no signs of encephalitis or CNS vasculitis, although the extent of neuroanatomic sampling was not provided31. A second study of COVID-19 autopsy findings included four brains that exhibited no encephalitis or neuronal necrosis, but mild hypoxemic changes in three of the four brains examined143. Although these studies have not identified specific neuropathologic alterations, the extent of involvement of the CNS in SARS-CoV-2 infection cannot be inferred from only 14 brains.
To establish how SARS-CoV-2 infection affects the CNS, the field will require detailed neuropathologic studies with thorough sampling of specific brain regions. At the Columbia University Medical Center, a current approach involves sampling of multiple neuroanatomic regions, including the cerebral cortex, watershed areas, white matter, olfactory system, hippocampus, amygdala, thalamus, hypothalamus, corpus striatum, pallidum, cerebellum, midbrain, pons, medulla oblongata, and cervical cord. We recommend that special attention be directed at documenting the presence and neuroanatomic distribution of hypoxia-related as well as inflammation-related pathologies, including leptomeningitis, encephalitis, and vasculitis.
The clinical perspective
Clinical implications of SARS-CoV-2 infection on PD are largely speculative apart from two case series and case reports45,46. A community-based case control study in Italy of 12 PD COVID-19 cases suggested substantial worsening of motor and non-motor symptoms during mild to moderate COVID-19 illness, independent of age and disease duration144, in line with an original case report series by Antonini et al. In another survey across the Lombardy region of Italy, 105 probable COVID-19 cases were identified and the authors concluded that the risk, morbidity, and mortality in patients with mild-to moderate PD with COVID-19 did not differ from the general population145. Several viewpoints and editorials have been published on the topic in addition to extensive coverage in social media and journal viewpoint papers146,147,148,149,150,151.
Currently there is no robust evidence that having PD imparts an increased risk for susceptibility to COVID-19 or that COVID-19 confers a greater risk of PD, although, as noted above, there are reported cases of worsening of PD symptoms in infected patients, particularly in older frail patients on advanced therapies and one case report of development of an acute hypokinetic syndrome with hyposmia post COVID-19.
Broadly, the clinical impact of COVID-19 on PD could occur through multiple avenues:
- 1.Development of COVID-19-related symptoms, particularly high fever, severe respiratory distress, coagulopathy-related syndrome, fatigue, myalgias, and related impaired stress mechanisms.
- 2.Worsening of pre-existing dyspnea due to respiratory distress; dyspnea may exist in up to 39% of PD patients152.
- 3.In acutely ill patients admitted to hospital, confusion and delirium could occur (reported in over 25% of COVID-19 hospitalized subjects out of a survey of 3500 patients)38.
- 4.Worsening of specific symptoms, including motor symptoms as well as non-motor issues, such as pain, anxiety, sleep disturbances and fatigue, especially with reduced access to physical therapy and counseling45,144.
- 5.Social isolation and aggravation of underlying cognitive and behavioral symptoms, specifically anxiety153.
- 6.Possibility of post-traumatic stress disorder (PTSD) as observed in previous SARS and MERS pandemics38.
- 7.Increased levodopa requirement during acute admissions and need for non-oral dopaminergic therapies in some subjects with severe COVID-19 related symptoms45.
- 8.Potential for drug interaction of over the counter cough remedies with anti-parkinsonian drugs such as monoamine oxidase inhibitors.
- 9.Complexity in therapeutic management related to limitations of in-person consultations and admissions to hospital147.
The impact of severe infection (by default, implying a high viral load or a pro-inflammatory state) may lead to hospitalization and the need for supported breathing or mechanical ventilation, particularly in older PD patients with multimorbidity and a high frailty index154. The issue is further compounded because such patients may be on non-oral therapies (subcutaneous apomorphine, intrajejunal levodopa infusion, and deep brain stimulation (DBS)) for advanced PD151. Limited observations from admission of such cases around the world (personal communication) and the published case series suggest that such patients are particularly vulnerable, with high mortality rates and may have an increased levodopa requirement during the acute illness45,46. Pre-existing dyspnea of PD152,155, respiratory muscle bradykinesia155 in addition to a possible direct SARS-CoV-2-related brainstem-generated suppression of cough reflex and perhaps of autoregulation of blood flow may play additional negative roles77,78,156,157.
Fatigue has been commonly reported after many viral infections, most notably with Epstein-Barr virus158, and is evident in many non-PD cases with COVID-19159. Fatigue was also common in the series of PD cases reported45 and is an important contributor of quality of life160. Myalgia is also common after viral illnesses including COVID-1940,161, and in some cases of COVID-19 with PD, myalgia can be severe and involve muscles of the back. If these observations are confirmed in larger cohorts of PD patients with COVID-19, specific anti-fatigue/myalgia measures may need to be implemented160. Consideration for the use of amantadine-like drugs may be particularly relevant given their putative antiviral effects162,163; however specific clinical trials are lacking.
Social isolation and its impact on PD are a concern and has been called a “hidden sorrow” of the pandemic164. Social isolation may cause heightened anxiety, aggravation of pre-existing depression, the negative effects of stress on PD165, as well as lack of exercise. In the previous SARS and MERS epidemics, one in three hospitalized cases went on to develop a PTSD with 15% developing depression and anxiety at 1 year, and fatigue in more than 15%38. Anxiety in PD during COVID-19-related lockdown and consequent stress is widely reported during telephone consultations in many countries, and specific strategies for home care using telemedicine or remote counselling may need to be implemented.
An overall consensus-led guideline for management of PD with varying grades of COVID-19 needs to be developed and circulated for implementation. A suggested template is provided in Fig. 2. These observations can be applied to the elderly as well as subjects with other neurodegenerative disorders, such as Alzheimer’s disease or amyotrophic lateral sclerosis.
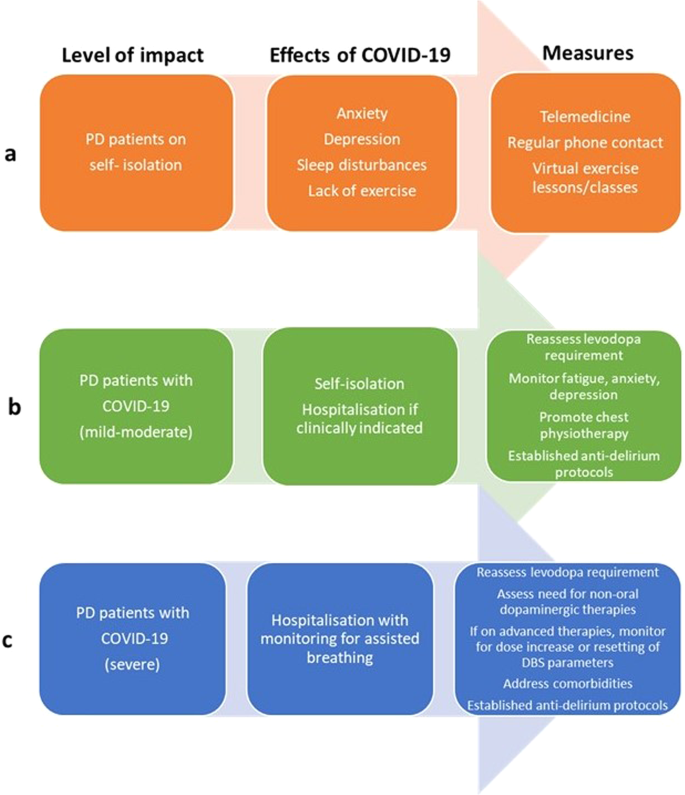
Conclusions
There has been a large number of papers on COVID-19 and PD speculating on etiology, risks and consequences, in addition to two documented case series of PD with COVID-19. We attempt to prove a critical approach to these observations from currently available clinical and molecular insights.
- The COVID-19 pandemic has led to an unprecedented crisis for older people globally. There is a broad range of COVID-19 symptoms, perhaps related to pre-existing conditions and in part to different modes of viral entry and the presence of T cells that are reactive to prior coronavirus infections. The neurological manifestations may be related to inflammation involving capillaries and the blood-brain barrier, hypoxemia, and thrombosis acting as triggers for seizures or leading to ischemic or hemorrhagic strokes.
- Neuropathology studies have not yet clearly answered the central issue of whether the virus enters central nervous system neurons, astrocytes or microglia.
- In brain vasculature, the cell types that express virus have not yet been identified.
- There is no clear evidence in human neurons or astrocytes for expression of the protein ACE2, which is thought to act as the major viral receptor that enables viral entry. Such expression may, however, be activated by inflammation, and thus comparison of healthy and infected brains will be important.
- There is a variety of alternative viral receptors for coronavirus, including sialic acid residues, that are insufficiently characterized and may provide entry into neurons and astrocytes.
- In contrast to the 1918 influenza pandemic and avian flu, reports of encephalopathy in COVID-19 have been slow to emerge, and there are so far no documented reports of an induction of parkinsonism apart from a single report. While a role for the virus in causing or exacerbating Parkinson’s disease appears unlikely at this time, the aggravation of specific motor and non-motor symptoms is reported.
- As the prevalence of PD rises sharply in the older age group, particularly in those over the age of 80 years, a personalized approach in the management of PD patients affected by COVID-19 based on clinical and basic science evidence is required. In addition, it will be important to monitor subjects after recovery, particularly for those with persisting hyposmia.
Data availability
Data sharing not applicable to this article as no data sets were generated or analyzed during the current study.
References
- Ye, Z. W. et al. Zoonotic origins of human coronaviruses. Int. J. Biol. Sci. 16, 1686–1697 (2020).CAS PubMed PubMed Central Google Scholar
- Kim, S. M., Kim, Y. I., Pascua, P. N. & Choi, Y. K. Avian influenza a viruses: evolution and zoonotic infection. Semin. Respir. Crit. Care. Med. 37, 501–511 (2016).PubMed PubMed Central Google Scholar
- Morens, D. M., Taubenberger, J. K. & Fauci, A. S. H7N9 avian influenza A virus and the perpetual challenge of potential human pandemicity. mBio 4. https://doi.org/10.1055/s-0036-1584953 (2013).
- Mostafa, A., Abdelwhab, E. M., Mettenleiter, T. C. & Pleschka, S. Zoonotic potential of influenza A Viruses: a comprehensive overview. Viruses. https://doi.org/10.3390/v10090497 (2018).
- Reperant, L. A., Kuiken, T., Osterhaus, A. D., Webby, R. J. & Webster, R. G. Influenza viruses: from birds to humans. Emergence of influenza A viruses. Hum. Vaccin. Immunother. 8, 7–16 (2012).PubMed Google Scholar
- Webster, R. G. Influenza virus: transmission between species and relevance to emergence of the next human pandemic. Arch. Virol. Suppl. 13, 105–113 (1997).CAS PubMed Google Scholar
- Nguyen, A. M. & Noymer, A. Influenza mortality in the United States, 2009 pandemic: burden, timing and age distribution. PLoS ONE 8, e64198 (2013).PubMed PubMed Central Google Scholar
- Salamatbakhsh, M., Mobaraki, K., Sadeghimohammadi, S. & Ahmadzadeh, J. The global burden of premature mortality due to the Middle East respiratory syndrome (MERS) using standard expected years of life lost, 2012 to 2019. BMC Public Health 19, 1523 (2019).PubMed PubMed Central Google Scholar
- Zheng, J. SARS-CoV-2: an emerging coronavirus that causes a global threat. Int. J. Biol. Sci. 16, 1678–1685 (2020).CAS PubMed PubMed Central Google Scholar
- Astuti, I. & Ysrafil Severe acute respiratory syndrome coronavirus 2 (SARS-CoV-2): an overview of viral structure and host response. Diabetes Metab. Syndr. 14, 407–412 (2020).PubMed PubMed Central Google Scholar
- Chen, N. et al. Epidemiological and clinical characteristics of 99 cases of 2019 novel coronavirus pneumonia in Wuhan, China: a descriptive study. Lancet 395, 507–513 (2020).CAS PubMed PubMed Central Google Scholar
- Grifoni, A. et al. Targets of T cell responses to SARS-CoV-2 coronavirus in humans with COVID-19 disease and unexposed individuals. Cell. https://doi.org/10.1016/j.cell.2020.05.015 (2020).
- Gu, J., Han, B. & Wang, J. COVID-19: gastrointestinal manifestations and potential fecal-oral transmission. Gastroenterology 158, 1518–1519 (2020).CAS PubMed Google Scholar
- Shahid, Z. et al. COVID-19 and older adults: what we know. J. Am. Geriatr. Soc. 68, 926–929 (2020).PubMed PubMed Central Google Scholar
- Mehndiratta, M. M., Mehndiratta, P. & Pande, R. Poliomyelitis: historical facts, epidemiology, and current challenges in eradication. Neurohospitalist 4, 223–229 (2014).PubMed PubMed Central Google Scholar
- O’Dowd, A. Covid-19: deaths in care home deaths in England and Wales rise sharply. BMJ (Clin. Res. ed.) 369, m1727 (2020).Google Scholar
- Abbatecola, A. M. & Antonelli-Incalzi, R. Editorial: COVID-19 spiraling of frailty in older Italian patients. J. Nutr. Health Aging 24, 453–455 (2020).CAS PubMed PubMed Central Google Scholar
- Wang, B., Li, R., Lu, Z. & Huang, Y. Does comorbidity increase the risk of patients with COVID-19: evidence from meta-analysis. Aging (Albany NY) 12, 6049–6057 (2020).CAS Google Scholar
- Garg, S. et al. Hospitalization rates and characteristics of patients hospitalized with laboratory-confirmed coronavirus disease 2019—COVID-NET, 14 States, March 1–30, 2020. MMWR Morb. Moral. Wkly Rep. 69, 458–464 (2020).CAS Google Scholar
- Miossec, P. Understanding the cytokine storm during COVID-19: contribution of preexisting chronic inflammation. Eur. J. Rheumatol. https://doi.org/10.5152/eurjrheum.2020.2062 (2020).
- Goh, K. J. et al. Rapid progression to acute respiratory distress syndrome: review of current understanding of critical illness from COVID-19 infection. Ann. Acad. Med. Singap. 49, 108–118 (2020).PubMed Google Scholar
- Harenberg, J. & Favaloro, E. COVID-19: progression of disease and intravascular coagulation – present status and future perspectives. Clin. Chem. Lab. Med. https://doi.org/10.1515/cclm-2020-0502 (2020).
- Levi, M., Thachil, J., Iba, T. & Levy, J. H. Coagulation abnormalities and thrombosis in patients with COVID-19. Lancet Haematol. https://doi.org/10.1016/s2352-3026(20)30145-9 (2020).
- Chiotos, K. et al. Multisystem inflammatory syndrome in children during the COVID-19 pandemic: a case series. J. Pediatric Infect. Dis. Soc. https://doi.org/10.1093/jpids/piaa069 (2020).
- Jose, R. J. & Manuel, A. COVID-19 cytokine storm: the interplay between inflammation and coagulation. Lancet Respir. Med. https://doi.org/10.1016/s2213-2600(20)30216-2 (2020).
- Mao, L. et al. Neurologic manifestations of hospitalized patients with coronavirus disease 2019 in Wuhan, China. JAMA Neurol. https://doi.org/10.1001/jamaneurol.2020.1127 (2020).
- Esposito, G. et al. Can the enteric nervous system be an alternative entrance door in SARS-CoV2 neuroinvasion? Brain. Behav. Immun. https://doi.org/10.1016/j.bbi.2020.04.060 (2020).
- Li, Y. C., Bai, W. Z. & Hashikawa, T. The neuroinvasive potential of SARS-CoV2 may play a role in the respiratory failure of COVID-19 patients. J. Med. Virol. https://doi.org/10.1002/jmv.25728 (2020).
- Moriguchi, T. et al. A first case of meningitis/encephalitis associated with SARS-Coronavirus-2. Int. J. Infect. Dis. 94, 55–58 (2020).CAS PubMed PubMed Central Google Scholar
- De Felice, F. G., Tovar-Moll, F., Moll, J., Munoz, D. P. & Ferreira, S. T. Severe acute respiratory syndrome coronavirus 2 (SARS-CoV-2) and the central nervous system. Trends Neurosci. https://doi.org/10.1016/j.tins.2020.04.004 (2020).
- Paniz-Mondolfi, A. et al. Central nervous system involvement by severe acute respiratory syndrome coronavirus-2 (SARS-CoV-2). J. Med. Virol. https://doi.org/10.1002/jmv.25915 (2020).
- Poyiadji, N. et al. COVID-19-associated acute hemorrhagic necrotizing encephalopathy: CT and MRI Features. Radiology. https://doi.org/10.1148/radiol.2020201187 (2020).
- Beyrouti, R. et al. Characteristics of ischaemic stroke associated with COVID-19. J. Neurol. Neurosurg. Psychiatry https://doi.org/10.1136/jnnp-2020-323586 (2020).
- Ebrille, E. et al. Syncope as the presenting symptom of COVID-19 infection. HeartRhythm Case Rep. https://doi.org/10.1016/j.hrcr.2020.04.015 (2020).
- Lechien, J. R. et al. Clinical and epidemiological characteristics of 1,420 European patients with mild-to-moderate coronavirus disease 2019. J. Intern. Med. https://doi.org/10.1111/joim.13089 (2020).
- Kotfis, K. et al. COVID-19: ICU delirium management during SARS-CoV-2 pandemic. Crit. Care 24, 176 (2020).PubMed PubMed Central Google Scholar
- Zanin, L. et al. SARS-CoV-2 can induce brain and spine demyelinating lesions. Acta Neurochir. (Wien). https://doi.org/10.1007/s00701-020-04374-x (2020).
- Rogers, J. P. et al. Psychiatric and neuropsychiatric presentations associated with severe coronavirus infections: a systematic review and meta-analysis with comparison to the COVID-19 pandemic. Lancet Psychiatry. https://doi.org/10.1016/S2215-0366(20)30203-0 (2020).
- Rábano-Suárez, P. et al. Generalized myoclonus in COVID-19. Neurology. https://doi.org/10.1212/wnl.0000000000009829 (2020).
- Wang, D. et al. Clinical characteristics of 138 hospitalized patients with 2019 novel coronavirus-infected pneumonia in Wuhan, China. JAMA 323, 1061–1069 (2020).CAS PubMed PubMed Central Google Scholar
- Toscano, G. et al. Guillain-Barré syndrome associated with SARS-CoV-2. N. Engl. J. Med. https://doi.org/10.1056/NEJMc2009191 (2020).
- D’Amico, F., Baumgart, D. C., Danese, S. & Peyrin-Biroulet, L. Diarrhea during COVID-19 infection: pathogenesis, epidemiology, prevention, and management. Clin. Gastroenterol. Hepatol. 18, 1663–1672 (2020).PubMed PubMed Central Google Scholar
- Menni, C. et al. Real-time tracking of self-reported symptoms to predict potential COVID-19. Nat. Med. https://doi.org/10.1038/s41591-020-0916-2 (2020).
- Paterson, R. W. et al. The emerging spectrum of COVID-19 neurology: clinical, radiological and laboratory findings. Brain. https://doi.org/10.1093/brain/awaa240 (2020).
- Antonini, A., Leta, V., Teo, J. & Chaudhuri, K. R. Outcome of Parkinson’s disease patients affected by COVID-19. Mov. Disord. https://doi.org/10.1002/mds.28104 (2020).
- Hainque, E., G., D. Rapid worsening in Parkinson’s disease may hide COVID-19 infection. Parkinsonism Relat. Disord. https://doi.org/10.1016/j.parkreldis.2020.05.008 (2020).
- Fazzini, E., Fleming, J. & Fahn, S. Cerebrospinal fluid antibodies to coronavirus in patients with Parkinson’s disease. Mov. Disord. 7, 153–158 (1992).CAS PubMed PubMed Central Google Scholar
- Arabi, Y. M. et al. Severe neurologic syndrome associated with Middle East respiratory syndrome corona virus (MERS-CoV). Infection 43, 495–501 (2015).CAS PubMed PubMed Central Google Scholar
- Yeh, E. A., Collins, A., Cohen, M. E., Duffner, P. K. & Faden, H. Detection of coronavirus in the central nervous system of a child with acute disseminated encephalomyelitis. Pediatrics 113, e73–e76 (2004).PubMed Google Scholar
- Nilsson, A., Edner, N., Albert, J. & Ternhag, A. Fatal encephalitis associated with coronavirus OC43 in an immunocompromised child. Infect. Dis. (Lond.) 52, 419–422 (2020).Google Scholar
- Jang, H., Boltz, D. A., Webster, R. G. & Smeyne, R. J. Viral parkinsonism. Biochim. Biophys. Acta 1792, 714–721 (2009).CAS PubMed Google Scholar
- Dourmashkin, R. R., Dunn, G., Castano, V. & McCall, S. A. Evidence for an enterovirus as the cause of encephalitis lethargica. BMC Infect. Dis. 12, 136 (2012).CAS PubMed PubMed Central Google Scholar
- Hoffman, L. A. & Vilensky, J. A. Encephalitis lethargica: 100 years after the epidemic. Brain 140, 2246–2251 (2017).PubMed Google Scholar
- Limphaibool, N., Iwanowski, P., Holstad, M. J. V., Kobylarek, D. & Kozubski, W. Infectious etiologies of parkinsonism: pathomechanisms and clinical implications. Front. Neurol. 10, 652 (2019).PubMed PubMed Central Google Scholar
- Henry, J., Smeyne, R. J., Jang, H., Miller, B. & Okun, M. S. Parkinsonism and neurological manifestations of influenza throughout the 20th and 21st centuries. Parkinsonism Relat. Disord. 16, 566–571 (2010).PubMed PubMed Central Google Scholar
- Houser, M. C. & Tansey, M. G. The gut-brain axis: is intestinal inflammation a silent driver of Parkinson’s disease pathogenesis? NPJ Parkinson’s Dis. 3, 3 (2017).Google Scholar
- Li, F. Structure, function, and evolution of coronavirus spike proteins. Annu. Rev. Virol. 3, 237–261 (2016).CAS PubMed PubMed Central Google Scholar
- Xu, X. et al. Evolution of the novel coronavirus from the ongoing Wuhan outbreak and modeling of its spike protein for risk of human transmission. Sci. China Life Sci. 63, 457–460 (2020).CAS PubMed PubMed Central Google Scholar
- Hoffmann, M. et al. SARS-CoV-2 cell entry depends on ACE2 and TMPRSS2 and is blocked by a clinically proven protease inhibitor. Cell 181, 271–280.e278 (2020).CAS PubMed PubMed Central Google Scholar
- Perrotta, F., Matera, M. G., Cazzola, M. & Bianco, A. Severe respiratory SARS-CoV2 infection: Does ACE2 receptor matter? Respir. Med. 168, 105996 (2020).PubMed PubMed Central Google Scholar
- Wang, Q. et al. Structural and functional basis of SARS-CoV-2 entry by using human ACE2. Cell. https://doi.org/10.1016/j.cell.2020.03.045 (2020).
- Magrone, T., Magrone, M. & Jirillo, E. Focus on receptors for coronaviruses with special reference to angiotensin-converting enzyme 2 as a potential drug Target—a perspective. Endocr. Metab. Immune Disord. Drug Targets. https://doi.org/10.2174/1871530320666200427112902 (2020).
- Wrapp, D. et al. Cryo-EM structure of the 2019-nCoV spike in the prefusion conformation. Science 367, 1260–1263 (2020).CAS PubMed PubMed Central Google Scholar
- Fantini, J., Di Scala, C., Chahinian, H. & Yahi, N. Structural and molecular modelling studies reveal a new mechanism of action of chloroquine and hydroxychloroquine against SARS-CoV-2 infection. Int. J. Antimicrob. Agents. https://doi.org/10.1016/j.ijantimicag.2020.105960 (2020).
- Milanetti, E., Miotto, M., Di Rienzo, L., Monti, M., Gosti, G. & Ruocco, G. In-Silico evidence for two receptors based strategy of SARS-CoV-2. bioRxiv. Preprint at https://doi.org/10.1101/2020.03.24.006197 (2020).
- He, X. et al. Temporal dynamics in viral shedding and transmissibility of COVID-19. Nat. Med. 26, 672–675. https://doi.org/10.1038/s41591-020-0869-5 (2020).Article CAS PubMed Google Scholar
- Robson, B. Bioinformatics studies on a function of the SARS-CoV-2 spike glycoprotein as the binding of host sialic acid glycans. Comput. Biol. Med. 122, 103849 (2020).CAS PubMed PubMed Central Google Scholar
- Wasik, B. R., Barnard, K. N. & Parrish, C. R. Effects of sialic acid modifications on virus binding and infection. Trends Microbiol. 24, 991–1001 (2016).CAS PubMed PubMed Central Google Scholar
- Albright, B. H., Simon, K. E., Pillai, M., Devlin, G. W. & Asokan, A. Modulation of sialic acid dependence influences the central nervous system transduction profile of adeno-associated viruses. J. Virol. https://doi.org/10.1128/jvi.00332-19 (2019).
- Jeffers, S. A. et al. CD209L (L-SIGN) is a receptor for severe acute respiratory syndrome coronavirus. Proc. Natl Acad. Sci. USA 101, 15748–15753 (2004).CAS PubMed PubMed Central Google Scholar
- Ding, Y. et al. Organ distribution of severe acute respiratory syndrome (SARS) associated coronavirus (SARS-CoV) in SARS patients: implications for pathogenesis and virus transmission pathways. J. Pathol. 203, 622–630 (2004).CAS PubMed PubMed Central Google Scholar
- Gu, J. et al. Multiple organ infection and the pathogenesis of SARS. J. Exp. Med. 202, 415–424 (2005).CAS Google Scholar
- Puelles, V. G. et al. Multiorgan and renal tropism of SARS-CoV-2. N. Engl. J. Med. https://doi.org/10.1056/NEJMc2011400 (2020).
- Wichmann, D. et al. Autopsy findings and venous thromboembolism in patients with COVID-19. Ann. Intern. Med. https://doi.org/10.7326/M20-2003 (2020)
- Sepehrinezhad, A., Shahbazi, A. & Negah, S. S. COVID-19 virus may have neuroinvasive potential and cause neurological complications: a perspective review. J. Neurovirol. https://doi.org/10.1007/s13365-020-00851-2 (2020).
- Natoli, S., Oliveira, V., Calabresi, P., Maia, L. F. & Pisani, A. Does SARS-Cov-2 invade the brain? Translational lessons from animal models. Eur. J. Neurol. https://doi.org/10.1111/ene.14277 (2020).
- Netland, J., Meyerholz, D. K., Moore, S., Cassell, M. & Perlman, S. Severe acute respiratory syndrome coronavirus infection causes neuronal death in the absence of encephalitis in mice transgenic for human ACE2. J. Virol. 82, 7264–7275 (2008).CAS PubMed PubMed Central Google Scholar
- Li, K. et al. Middle East respiratory syndrome coronavirus causes multiple organ damage and lethal disease in mice transgenic for human dipeptidyl peptidase 4. J. Infect. Dis. 213, 712–722 (2016).CAS PubMed Google Scholar
- Li, Y. C., Bai, W. Z., Hirano, N., Hayashida, T. & Hashikawa, T. Coronavirus infection of rat dorsal root ganglia: ultrastructural characterization of viral replication, transfer, and the early response of satellite cells. Virus Res. 163, 628–635 (2012).CAS PubMed PubMed Central Google Scholar
- Li, Y. C. et al. Neurotropic virus tracing suggests a membranous-coating-mediated mechanism for transsynaptic communication. J. Comp. Neurol. 521, 203–212 (2013).CAS PubMed Google Scholar
- Andries, K. & Pensaert, M. B. Immunofluorescence studies on the pathogenesis of hemagglutinating encephalomyelitis virus infection in pigs after oronasal inoculation. Am. J. Vet. Res. 41, 1372–1378 (1980).CAS PubMed Google Scholar
- Chasey, D. & Alexander, D. J. Morphogenesis of avian infectious bronchitis virus in primary chick kidney cells. Arch. Virol. 52, 101–111 (1976).CAS PubMed PubMed Central Google Scholar
- Pan, W. et al. Cytokine signaling modulates blood-brain barrier function. Curr. Pharm. Des. 17, 3729–3740 (2011).CAS PubMed PubMed Central Google Scholar
- Obermeier, B., Daneman, R. & Ransohoff, R. M. Development, maintenance and disruption of the blood-brain barrier. Nat. Med. 19, 1584–1596 (2013).CAS PubMed PubMed Central Google Scholar
- Muldoon, L. L. et al. Immunologic privilege in the central nervous system and the blood-brain barrier. J. Cereb. Blood. Flow. Metab. 33, 13–21 (2013).CAS PubMed Google Scholar
- Pilotto, A. et al. Steroid-responsive encephalitis in Covid-19 disease. Ann. Neurol. https://doi.org/10.1002/ana.25783 (2020).
- Tipnis, S. R. et al. A human homolog of angiotensin-converting enzyme. Cloning and functional expression as a captopril-insensitive carboxypeptidase. J. Biol. Chem. 275, 33238–33243 (2000).CAS PubMed Google Scholar
- Jia, H. P. et al. Ectodomain shedding of angiotensin converting enzyme 2 in human airway epithelia. Am. J. Physiol. Lung Cell Mol. Physiol. 297, L84–L96 (2009).CAS PubMed PubMed Central Google Scholar
- Xiao, F. et al. Characterization of angiotensin-converting enzyme 2 ectodomain shedding from mouse proximal tubular cells. PLoS ONE 9, e85958 (2014).PubMed PubMed Central Google Scholar
- Li, M. Y., Li, L., Zhang, Y. & Wang, X. S. Expression of the SARS-CoV-2 cell receptor gene ACE2 in a wide variety of human tissues. Infect. Dis. Poverty 9, 45 (2020).PubMed PubMed Central Google Scholar
- Sluimer, J. C. et al. Angiotensin-converting enzyme 2 (ACE2) expression and activity in human carotid atherosclerotic lesions. J. pathol. 215, 273–279 (2008).CAS PubMed Google Scholar
- Hamming, I. et al. Tissue distribution of ACE2 protein, the functional receptor for SARS coronavirus. A first step in understanding SARS pathogenesis. J. pathol. 203, 631–637 (2004).CAS PubMed PubMed Central Google Scholar
- Doobay, M. F. et al. Differential expression of neuronal ACE2 in transgenic mice with overexpression of the brain renin-angiotensin system. Am. J. Physiol. Regul. Integr. Comp. Physiol. 292, R373–R381 (2007).CAS PubMed Google Scholar
- Gowrisankar, Y. V. & Clark, M. A. Angiotensin II regulation of angiotensin-converting enzymes in spontaneously hypertensive rat primary astrocyte cultures. J. Neurochem. 138, 74–85 (2016).CAS PubMed Google Scholar
- Xia, H. & Lazartigues, E. Angiotensin-converting enzyme 2: central regulator for cardiovascular function. Curr. Hypertens. Rep. 12, 170–175 (2010).CAS PubMed PubMed Central Google Scholar
- Xia, H., Sriramula, S., Chhabra, K. H. & Lazartigues, E. Brain angiotensin-converting enzyme type 2 shedding contributes to the development of neurogenic hypertension. Circ. res. 113, 1087–1096 (2013).CAS PubMed PubMed Central Google Scholar
- Martin, D., Xu, J., Porretta, C. & Nichols, C. D. Neurocytometry: flow cytometric sorting of specific neuronal populations from human and rodent brain. ACS Chem. Neurosci. 8, 356–367 (2017).CAS PubMed Google Scholar
- Zhang, R. et al. Role of HIF-1alpha in the regulation ACE and ACE2 expression in hypoxic human pulmonary artery smooth muscle cells. Am. J. Physiol. Lung Cell Mol. Physiol. 297, L631–L640 (2009).CAS PubMed Google Scholar
- Ziegler, C. G. K. et al. SARS-CoV-2 Receptor ACE2 Is an Interferon-Stimulated Gene in Human Airway Epithelial Cells and Is Detected in Specific Cell Subsets across Tissues. Cell. https://doi.org/10.1016/j.cell.2020.04.035 (2020).
- Xu, J., Sriramula, S. & Lazartigues, E. Excessive glutamate stimulation impairs ACE2 activity through ADAM17-mediated shedding in cultured cortical neurons. Cell Mol. Neurobiol. 38, 1235–1243 (2018).CAS PubMed Google Scholar
- Chen, R. et al. The spatial and cell-type distribution of SARS-CoV-2 receptor ACE2 in human and mouse brain. bioRxiv, 2020.2004.2007.030650. Preprint at https://doi.org/10.1101/2020.04.07.030650 (2020).
- Muus, C. et al. Integrated analyses of single-cell atlases reveal age, gender, and smoking status associations with cell type-specific expression of mediators of SARS-CoV-2 viral entry and highlights inflammatory programs in putative target cells. bioRxiv, 2020.2004.2019.049254. Preprint at https://doi.org/10.1101/2020.04.19.049254 (2020).
- Gordon, D. E. et al. A SARS-CoV-2 protein interaction map reveals targets for drug repurposing. Nature. https://doi.org/10.1038/s41586-020-2286-9 (2020).
- Lippi, A., Domingues, R., Setz, C., Outeiro, T. F. & Krisko, A. SARS-CoV-2: at the crossroad between aging and neurodegeneration. Mov. Disord. https://doi.org/10.1002/mds.28084 (2020).
- Ezzat, K. et al. The viral protein corona directs viral pathogenesis and amyloid aggregation. Nat. Commun. 10, 2331 (2019).
- Tulisiak, C. T., Mercado, G., Peelaerts, W., Brundin, L. & Brundin, P. Can infections trigger alpha-synucleinopathies? Prog. Mol. Biol. Transl. Sci. 168, 299–322 (2019).CAS PubMed PubMed Central Google Scholar
- Massey, A. R. & Beckham, J. D. Alpha-synuclein, a novel viral restriction factor hiding in plain sight. DNA Cell. Biol. 35, 643–645 (2016).CAS PubMed Google Scholar
- Lechien, J. R. et al. Olfactory and gustatory dysfunctions as a clinical presentation of mild-to-moderate forms of the coronavirus disease (COVID-19): a multicenter European study. Eur. Arch. Otorhinolaryngol. https://doi.org/10.1007/s00405-020-05965-1 (2020).
- Heinzel, S. et al. Update of the MDS research criteria for prodromal Parkinson’s disease. Mov. Disord. 34, 1464–1470 (2019).PubMed Google Scholar
- Kotecha, A. M., Corrêa, A. D. C., Fisher, K. M. & Rushworth, J. V. Olfactory dysfunction as a global biomarker for sniffing out Alzheimer’s disease: a meta-analysis. Biosensors. https://doi.org/10.3390/bios8020041 (2018).
- Barresi, M. et al. Evaluation of olfactory dysfunction in neurodegenerative diseases. J. Neurol. Sci. 323, 16–24 (2012).PubMed Google Scholar
- Abderrahmane, A. et al. Can the 2019 novel coronavirus cause Parkinson’s disease? Mov. Disord. https://doi.org/10.1002/mds.28118 (2020).
- Giacomelli, A. et al. Self-reported olfactory and taste disorders in SARS-CoV-2 patients: a cross-sectional study. Clin. Infect. Dis. https://doi.org/10.1093/cid/ciaa330 (2020).
- Lovato, A. & de Filippis, C. Clinical presentation of COVID-19: a systematic review focusing on upper airway symptoms. Ear Nose Throat J. https://doi.org/10.1177/0145561320920762 (2020).
- Ponsen, M. M. et al. Idiopathic hyposmia as a preclinical sign of Parkinson’s disease. Ann. Neurol. 56, 173–181 (2004).PubMed Google Scholar
- Haddadi, K., Ghasemian, R. & Shafizad, M. Basal ganglia involvement and altered mental status: a unique neurological manifestation of coronavirus disease 2019. Cureus 12, e7869 (2020).PubMed PubMed Central Google Scholar
- Guttman, M. Receptors in the basal ganglia. Can. J. Neurol. Sci. 14, 395–401 (1987).CAS PubMed Google Scholar
- Goldstein, D. S. Dysautonomia in Parkinson disease. Compr. Physiol. 4, 805–826 (2014).PubMed PubMed Central Google Scholar
- Méndez-Guerrero, A. et al. Acute hypokinetic-rigid syndrome following SARS-CoV-2 infection. Neurology. https://doi.org/10.1212/wnl.0000000000010282 (2020).
- Rodriguez-Perez, A. I. et al. Angiotensin type 2 receptors: Role in aging and neuroinflammation in the substantia nigra. Brain. Behav. Immun. https://doi.org/10.1016/j.bbi.2019.12.011 (2019).
- Joglar, B. et al. The inflammatory response in the MPTP model of Parkinson’s disease is mediated by brain angiotensin: relevance to progression of the disease. J. Neurochem. 109, 656–669 (2009).CAS PubMed Google Scholar
- Garretti, F., Agalliu, D., Lindestam Arlehamn, C. S., Sette, A. & Sulzer, D. Autoimmunity in Parkinson’s Disease: the role of α-synuclein-specific T cells. Front. Immunol. 10, 303 (2019).CAS PubMed PubMed Central Google Scholar
- Lindestam Arlehamn, C. S., Garretti, F., Sulzer, D. & Sette, A. Roles for the adaptive immune system in Parkinson’s and Alzheimer’s diseases. Curr. Opin. Immunol. 59, 115–120 (2019).CAS PubMed Google Scholar
- Block, M. L., Zecca, L. & Hong, J. S. Microglia-mediated neurotoxicity: uncovering the molecular mechanisms. Nat. Rev. Neurosci. 8, 57–69 (2007).CAS PubMed Google Scholar
- Williams, G. P. et al. T cell infiltration in both human multiple system atrophy and a novel mouse model of the disease. Acta Neuropathol. 139, 855–874 (2020).CAS PubMed PubMed Central Google Scholar
- Lodygin, D. et al. β-Synuclein-reactive T cells induce autoimmune CNS grey matter degeneration. Nature 566, 503–508 (2019).CAS PubMed Google Scholar
- Gagne, J. J. & Power, M. C. Anti-inflammatory drugs and risk of Parkinson disease: a meta-analysis. Neurology 74, 995–1002 (2010).CAS PubMed PubMed Central Google Scholar
- Jang, H. et al. Highly pathogenic H5N1 influenza virus can enter the central nervous system and induce neuroinflammation and neurodegeneration. Proc. Natl Acad. Sci. USA 106, 14063–14068 (2009).CAS PubMed PubMed Central Google Scholar
- Jang, H. et al. Inflammatory effects of highly pathogenic H5N1 influenza virus infection in the CNS of mice. J. Neurosci. 32, 1545–1559 (2012).CAS PubMed PubMed Central Google Scholar
- Bantle, C. M. et al. Infection with mosquito-borne alphavirus induces selective loss of dopaminergic neurons, neuroinflammation and widespread protein aggregation. NPJ Parkinsons Dis. 5, 20 (2019).PubMed PubMed Central Google Scholar
- Kobasa, D. et al. Enhanced virulence of influenza A viruses with the haemagglutinin of the 1918 pandemic virus. Nature 431, 703–707 (2004).CAS PubMed Google Scholar
- Kobasa, D. et al. Aberrant innate immune response in lethal infection of macaques with the 1918 influenza virus. Nature 445, 319–323 (2007).CAS PubMed Google Scholar
- Miyamoto, K. et al. Systemic inflammatory response syndrome and prolonged hypoperfusion lesions in an infant with respiratory syncytial virus encephalopathy. J. Infect. Chemother. 19, 978–982 (2013).PubMed PubMed Central Google Scholar
- Sadasivan, S., Sharp, B., Schultz-Cherry, S. & Smeyne, R. Synergistic effects of influenza and 1-methyl-4-phenyl-1,2,3,6-tetrahydropyridine (MPTP) can be eliminated by the use of influenza therapeutics: experimental evidence for the multi-hit hypothesis. NPJ Parkinsons Dis. https://doi.org/10.1038/s41531-017-0019-z (2017).
- Banks, W. A., Kastin, A. J. & Broadwell, R. D. Passage of cytokines across the blood-brain barrier. Neuroimmunomodulation 2, 241–248 (1995).CAS PubMed Google Scholar
- Mohanty, S. et al. Prolonged proinflammatory cytokine production in monocytes modulated by interleukin 10 after influenza vaccination in older adults. J. Infect. Dis. 211, 1174–1184 (2015).CAS PubMed Google Scholar
- Bird, N. L. et al. Oseltamivir prophylaxis reduces inflammation and facilitates establishment of cross-strain protective T cell memory to influenza viruses. PLoS ONE 10, e0129768 (2015).PubMed PubMed Central Google Scholar
- Varga, Z. et al. Endothelial cell infection and endotheliitis in COVID-19. Lancet 395, 1417–1418 (2020).CAS PubMed PubMed Central Google Scholar
- Barton, L. M., Duval, E. J., Stroberg, E., Ghosh, S. & Mukhopadhyay, S. COVID-19 autopsies, Oklahoma, USA. Am. J. Clin. Pathol. 153, 725–733 (2020).CAS PubMed Google Scholar
- Xu, Z. et al. Pathological findings of COVID-19 associated with acute respiratory distress syndrome. Lancet Respir. Med. 8, 420–422 (2020).CAS PubMed PubMed Central Google Scholar
- Tian, S. et al. Pulmonary pathology of early-phase 2019 novel coronavirus (COVID-19) pneumonia in two patients with lung cancer. J. Thorac. Oncol. 15, 700–704 (2020).CAS PubMed PubMed Central Google Scholar
- Adachi, T. et al. Clinicopathologic and immunohistochemical findings from autopsy of patient with COVID-19, Japan. Emerg. Infect. Dis. https://doi.org/10.3201/eid2609.201353 (2020).
- Menter, T. et al. Post-mortem examination of COVID19 patients reveals diffuse alveolar damage with severe capillary congestion and variegated findings of lungs and other organs suggesting vascular dysfunction. Histopathology. https://doi.org/10.1111/his.14134 (2020).
- Cilia, R. et al. Effects of COVID-19 on Parkinson’s disease clinical features: a community-based case-control study. Mov. Disord. https://doi.org/10.1002/mds.28170 (2020).
- Fasano, A. et al. COVID-19 in Parkinson’s disease patients living in Lombardy, Italy. Mov. Disord. https://doi.org/10.1002/mds.28176 (2020).
- Papa, S. M. et al. Impact of the COVID-19 pandemic on Parkinson’s disease and movement disorders. Mov. Disord. https://doi.org/10.1002/mds.28067 (2020).
- Stoessl, A. J., Bhatia, K. P. & Merello, M. Movement disorders in the world of COVID-19. Mov. Disord. Clin. Pract. 7, 355–356 (2020).PubMed PubMed Central Google Scholar
- Bhidayasiri, R., Virameteekul, S., Kim, J. M., Pal, P. K. & Chung, S. J. COVID-19: an early review of its global impact and considerations for Parkinson’s Disease Patient Care. J. Mov. Disord. https://doi.org/10.14802/jmd.20042 (2020).
- Schirinzi, T. et al. Self-reported needs of patients with Parkinson’s disease during COVID-19 emergency in Italy. Neurol. Sci. https://doi.org/10.1007/s10072-020-04442-1 (2020).
- Tipton, P. W. & Wszolek, Z. K. What can Parkinson’s disease teach us about COVID-19? Neurol. Neurochir. Pol. 54, 204–206 (2020).PubMed Google Scholar
- Fasano, A. et al. Management of advanced therapies in Parkinson’s disease patients in times of humanitarian crisis: the COVID-19 experience. Mov. Disord. Clin. Pract. 7, 361–372 (2020).PubMed PubMed Central Google Scholar
- Baille, G. et al. Dyspnea: an underestimated symptom in Parkinson’s disease. Parkinsonism Relat. Disord. 60, 162–166 (2019).PubMed Google Scholar
- Prasad, S. et al. Parkinson’s Disease and COVID-19: perceptions and implications in patients and caregivers. Mov. Disord. https://doi.org/10.1002/mds.28088 (2020).
- Roland, K. P., Cornett, K. M., Theou, O., Jakobi, J. M. & Jones, G. R. Concurrence of Frailty and Parkinson’s disease. J. Frailty Aging 1, 123–127 (2012).CAS PubMed Google Scholar
- Torsney, K. M. & Forsyth, D. Respiratory dysfunction in Parkinson’s disease. J. R. Coll. Physicians Edinb. 47, 35–39 (2017).CAS PubMed Google Scholar
- McCray, P. B. Jr. et al. Lethal infection of K18-hACE2 mice infected with severe acute respiratory syndrome coronavirus. J. Virol. 81, 813–821 (2007).CAS PubMed Google Scholar
- Matsuda, K. et al. The vagus nerve is one route of transneural invasion for intranasally inoculated influenza a virus in mice. Vet. Pathol. 41, 101–107 (2004).CAS PubMed Google Scholar
- Jones, J. F. Epstein-Barr virus and the chronic fatigue syndrome: a short review. Microbiol. Sci. 5, 366–369 (1988).CAS PubMed Google Scholar
- Guan, W. J. et al. Clinical characteristics of coronavirus disease 2019 in China. N. Engl. J. Med. 382, 1708–1720 (2020).CAS PubMed Google Scholar
- Lazcano-Ocampo, C. et al. Identifying and responding to fatigue and apathy in Parkinson’s disease: a review of current practice. Expert Rev. Neurother. 20, 477–495 (2020).CAS PubMed Google Scholar
- Huang, C. et al. Clinical features of patients infected with 2019 novel coronavirus in Wuhan, China. Lancet 395, 497–506 (2020).CAS PubMed PubMed Central Google Scholar
- Smieszek, S. P., Przychodzen, B. P. & Polymeropoulos, M. H. Amantadine disrupts lysosomal gene expression: a hypothesis for COVID19 treatment. Int. J. Antimicrob. Agents. https://doi.org/10.1016/j.ijantimicag.2020.106004 (2020).
- Martinez-Martin, P. et al. Impact of fatigue in Parkinson’s disease: the fatigue impact scale for daily use (D-FIS). Qual. Life Res. 15, 597–606 (2006).